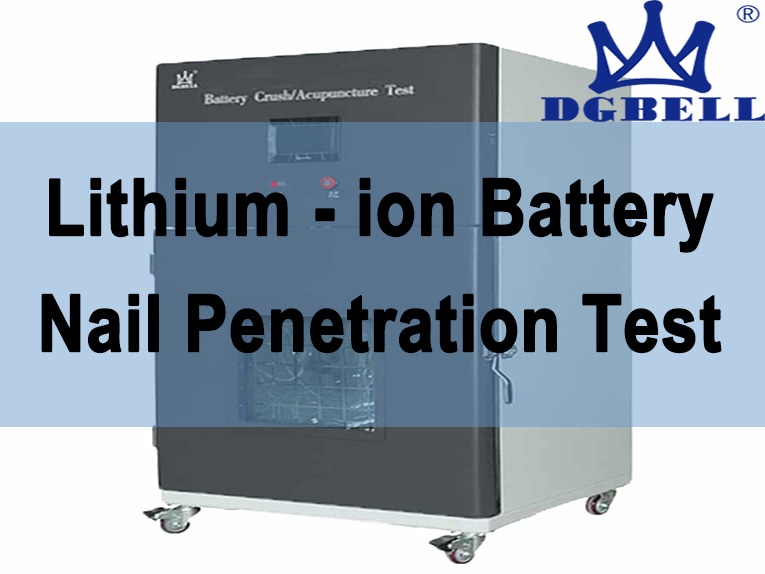
#Industry News
Lithium-ion Battery Nail Penetration Safety Test – Part 1
Lithium-ion Battery Nail Penetration Safety Test – Part 1
The compression of power batteries by sharp objects is the main form of damage caused by car collisions, and it is also a very severe working condition. In severe cases, lithium-ion batteries can explode, causing damage to electric vehicles and even personal injury.
In order to reveal the safety performance of lithium-ion batteries under nail penetration conditions, this test used the DGBELL nail penetration test chamber, and used a 5mm diameter flat head tungsten steel needle to pierce the 18650 cylindrical lithium-ion battery.
The influence of four parameters (state of charge, nail penetration speed, depth, and position) on the safety performance of lithium-ion batteries was discussed, and the phenomenon of thermal runaway of lithium-ion batteries was observed. The characterization data of temperature, open circuit voltage, and load of lithium-ion batteries before and after the test were recorded. The testal results show that lithium-ion batteries exhibit significant evolution patterns under needle puncture conditions.
Lithium ion batteries do not immediately experience thermal runaway after needle puncture failure, but rather have a certain reaction time; The higher the state of charge, the deeper the needle penetration, and the more likely lithium-ion batteries are to experience thermal runaway, which is positively correlated with the severity of thermal runaway;
The closer it is to the positive and negative terminals of a lithium-ion battery, the more intense the reaction becomes; There is no significant correlation between acupuncture speed and whether thermal runaway occurs. Finally, based on the testal results, suggestions were provided for the transportation, safe use, and early warning algorithm design of lithium-ion battery packs.
1 Test Introduction
The test uses 18650 cylindrical lithium-ion batteries with a rated capacity of 1200 m Ah. Use a battery testing system to charge and discharge lithium batteries to the required SOC in the test using a constant current and constant voltage method.
In the test, the range is 0-200mm and the force value is 2-20 kN to simulate the process of needle punching loading. Use a flat headed solid tungsten steel needle with a diameter of 5 mm. Considering the risk of explosion of lithium batteries during needle punching loading, the test chamber has explosion-proof function.
The test considered the influence of four factors: SOC, speed, position, and depth, including four sets of tests. In the testal test, the steel needles were inserted at three locations, namely at the top, middle, and bottom of the lithium battery. After piercing the battery with a steel needle, observe for 1 hour and then remove the battery to save the current testal data. After each test, let it sit for 30 minutes to check the temperature inside the steel needle and test chamber.
Wait until it returns to room temperature before proceeding to the next test. In addition, all testal conditions were controlled using the method of controlling variables to exclude the influence of unrelated factors. And each test should be repeated at least 3 times, and the data from the test with good repeatability should be taken for research to eliminate the randomness of the test and ensure the accuracy of the testal data.
2 Nail penetration test results and analysis
2.1 Characteristics analysis
Five different states of charge (SOC of 20%, 40%, 60%, 80%, and 100%) of lithium batteries were selected for needle puncture tests. Record the ultimate load and terminal voltage drop time nodes of lithium batteries under different SOC conditions.
The trend in the front section of the curve under the five states of charge is roughly the same, first experiencing a linear growth stage, then entering a slow growth platform area with little change in carrying capacity, and then entering a high-speed growth stage, showing exponential growth. In the early stage of testal loading, the steel shell of the battery begins to bear the load first, and after a certain degree of continuous loading, it then begins to compress the gaps between the inner core layers and the innermost hollow part. So the initial load change is very small, and after the internal gaps of the battery are compacted, the load increases rapidly. The load generated at the moment when the strength of the battery casing fails and is punctured is called the ultimate load.
Through tests, it can be seen that there is not a simple growth relationship between ultimate load and SOC, and the maximum ultimate load corresponds to SOC of 60%. The possible reason is that the amount of lithium embedded in the negative electrode material increases with the increase of SOC, so batteries with 60% SOC have stronger load-bearing capacity compared to batteries with 60% SOC or below. When the SOC is greater than 60%, the content of active chemicals inside the lithium battery gradually increases.
During the needle punching process, the thermal runaway reaction is severe, and a large amount of gas overflows from the positive safety valve and needle punching position, causing the lithium battery to swell and slide limitedly, resulting in a decrease in its reaction force. Therefore, the change in lithium insertion amount and the occurrence of limited sliding at this time will lead to a decrease in its ultimate load.
In order to eliminate testal randomness, Group 1 conducted multiple repeated tests at each state of charge, and it can be seen that the average ultimate load of the battery is not simply a growth relationship with SOC. When the SOC is between 20% and 60%, the average ultimate load of lithium batteries increases with the increase of SOC, and the average ultimate load of lithium batteries with an SOC of 60% is greater than 80%. When the SOC is between 80% and 100%, it also increases with the increase of SOC.
Record the voltage time curves of different SOC batteries. The results indicate that the SOC of the battery has a certain impact on its voltage response, and the time point when the battery terminal voltage drops is highly consistent with the time point when the battery reaches its maximum load, indicating that the internal short circuit of the battery occurs at the moment of puncture.
The voltage with SOC of 20% and 40% did not drop sharply to 0 V at the time node of the maximum load drop, but first experienced a period of fluctuation and then dropped to around 0 V. The reason for this may be that the chemical reactivity inside the battery decreases under low SOC conditions, and no immediate reaction occurs during a short circuit.
After comparing the temperature time curves under different SOC conditions, it can be found that the higher the state of charge of the battery, the earlier the temperature rise, and the corresponding peak temperature is also higher. On the other hand, high SOC lithium batteries have a high content of internal chemicals, and the reaction is intense when a steel needle is inserted into the battery for short circuit. Lithium batteries have all experienced thermal runaway, with electrolyte flowing out from the puncture site and emitting a large amount of white smoke after deformation.
In the later stage of loading, the battery temperature rapidly increases in a short period of time, accompanied by the rapid discharge of irritating gases. This is because after the steel needle pierces the lithium battery shell, the internal active substances and electrolyte undergo oxidation-reduction reactions with air.
The deformation after needle puncture at 100% SOC resulted in an explosion after approximately 136 seconds of loading. The positive safety valve was ejected, emitting a large amount of white smoke, and the highest temperature reached 162.9 ℃ at the moment of explosion. Due to a large amount of gas escaping from the puncture site at the moment of explosion, it can be seen that the battery puncture site is severely damaged and swollen.